Chapter 6
What we found: Metabolism
What you’ll learn in this chapter
In this chapter, we’ll cover:
- What metabolism is, and what helps regulate it;
- How vitamin D plays a role, and how genetic variations might affect vitamin D metabolism;
- Thyroid function and the shared genetic roots of autoimmune diseases;
- The role that genes may play in regulating glucose / insulin, blood lipids, and metabolic syndrome;
- Whether genes can predict risks for Alzheimer’s disease; and
- What you can learn about all of this through genetic testing.
Two important points to keep in mind:
- While science is cool, and we have some interesting genetic findings and areas for further exploration, we still know comparatively very little.
- Just because a genetic test can tell you what factors might affect your metabolic health, it doesn’t mean that it can tell you the “perfect” diet, exercise, or supplement plan for you.
As you read this chapter, remember our usual caution:
As with most preferences, health risks, and genetic traits, there are many complex, interrelated factors.
There is almost never one single gene that inevitably leads to a given result.
Any genetic data we share are simply clues for further exploration.
What is “metabolism”?
Life is constant change.
The term “metabolism” comes to us from the ancient Greek metabolē, or “change”, and it refers to all the coordinated, dynamic processes that must take place for us to stay alive. These processes include absorbing, transporting and using nutrients or synthesizing substances we need.
By way of the proteins they code for, genes affect all metabolic processes, such as:
- growth, tissue recovery and repair;
- processing nutrients and transporting them into cells;
- synthesizing hormones and other cell signaling molecules;
- establishing basal metabolic rate (aka our “idling speed”); and
- how well we digest, absorb, use, and/or store any nutrients we eat.
Genes are involved in many ways.
For instance:
- Genes may directly act on these metabolic processes — for example, by coding for a cellular protein that controls how nutrients get transported into that cell.
- Genes may affect the activity of other genes.
- Genes may have a strong effect, a weak effect, or no effect at all… unless other factors are present. Sometimes seemingly unrelated genes must be co-transcribed to have a physiological impact.
We could write an entire textbook (or ten) about metabolism, and how genes may affect it.
For this chapter, we’ll focus on a few key indicators that may affect your metabolic health, fitness, and function.
In the next chapter, we’ll look at what affects your body weight and composition.
What regulates metabolism?
Mission Control: the hypothalamus
Deep in your brain is an almond-sized nugget known as the hypothalamus. This gland is tiny but powerful: It regulates many of your body’s metabolic functions.
The hypothalamus bridges the endocrine (organ-to-organ signaling) system and the nervous system: It interprets chemical messages and information from both inner physiological processes and the outside world into “what to do next”.
In particular, the hypothalamus helps maintain homeostasis by interpreting the body’s actions and reactions and initiating responses to keep things in equilibrium.
For instance, the hypothalamus helps to regulate:
- blood pressure and heart rate;
- body temperature;
- fluid-salt (aka electrolyte) balance;
- appetite / hunger and thirst;
- basal metabolic rate (BMR), the body’s “idling speed”;
- body weight and energy storage;
- digestion and gastric motility; and
- sleep and wakefulness.
The hypothalamus monitors and responds to signals from inside the body as well as the outside world, such as:
- how much energy we have available or stored (e.g., in the form of body fat or circulating glucose);
- inputs from our autonomic nervous system (ANS), which is the nervous system that controls internal organs such as our heart, lungs, or digestive tract;
- light and dark (which help cue sleep cycles);
- temperature outside and inside our bodies;
- scents and other volatile chemicals; or
- pathogens such as bacteria or viruses.
As the hypothalamus gathers environmental information from external and internal cues, it sends chemical messages “downstream” to other endocrine glands, such as the pituitary.
Peripheral regulation: sensors and storage
Along with central regulation of our metabolism, we are always gathering information from physiological processes that are happening peripherally — in other parts of the body besides the brain, such as our:
- sensory organs (e.g., eyes, skin, taste buds, etc.);
- vestibular (balance-sensing) and proprioceptive (position- and movement-sensing) systems;
- other endocrine organs (e.g. thyroid, adrenals, gonads, etc.);
- digestive organs;
- innate and adaptive immune systems; and
- energy storage depots (such as our stored body fat or stored liver glycogen).
Feedback loops and signaling pathways
We regulate our metabolism via a series of complex feedback loops, a cycle where the output of a process is used as input for the next step.
For instance, if our hypothalamus senses we are getting low on stored energy (perhaps because blood glucose drops, or our body fat stores are getting low), it triggers our appetite centers, pushing us to eat.
Conversely, if the hypothalamus senses we have excess energy available, it might store that energy, use it to drive metabolic functions, or burn it off as heat.
You can think of genetic expression as part of these feedback loops: When stuff happens, it triggers other stuff to happen (or stop happening).
Gene expression and epigenetic regulation control what gets turned on or off; what gets enabled or inhibited. The proteins that genes produce respond to one another, or what substances are circulating nearby, or environmental factors.
Cells secrete and receive molecules, which help them communicate with signaling pathways. These are a series of interactions that trigger each other in a step-by-step process.
Imagine a courier delivering a package to the front desk of an office. The receptionist receives the package, then calls someone else to let them know the package has been delivered.
The same idea works here.
Most often, a substance (known as a first messenger) will bind to a receptor on a cell membrane. This binding sends signals — known as second messengers — elsewhere in the cell to do a specific task. This whole process is known as signal transduction.
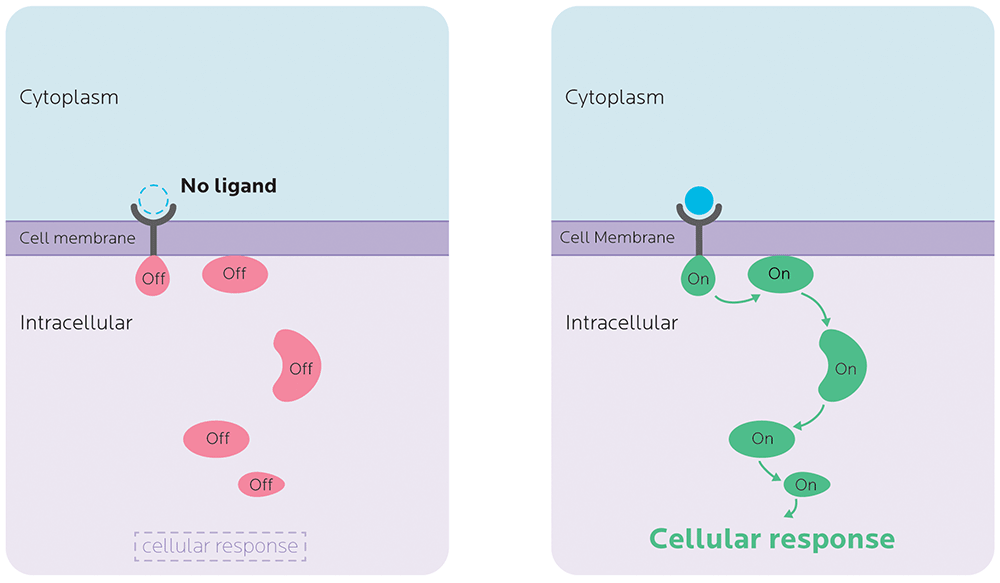
Many of the genes that we’ll talk about code for mRNAs that make messenger proteins — in other words, essential parts of metabolic processes. Variations in the expression of genes that code for these proteins can affect, for example, whether the signal even makes it to the cell, whether the cell accepts the signal, whether the first messenger is able to communicate with the second one, and so forth.
You can’t change your genes.
But you can affect these signaling pathways with your lifestyle choices and environment.
The genetics of metabolism
When you consider all the moving parts of metabolism, it’s easy to imagine how genetic factors might play significant and complex roles.
In this chapter, we’ll look at a few biological markers and processes to see how your genes may play a role, and what you might learn via genetic testing.
Vitamin D
Looking at vitamin D helps us understand some of the mechanisms by which genetic expression and regulation can affect our metabolism, which can, in turn, affect how we respond to stimuli like food and exercise.
Vitamin D is the name we give to a group of fat-soluble secosteroids. Steroid molecules have a variety of functions in our bodies (for instance, our sex hormones are also steroids). We’ll look more at steroid molecules below when we look at cholesterol.
We can get vitamin D from food. Vitamin D3 (cholecalciferol) is found in animal foods like oily fish, egg yolk, liver and milk. Vitamin D2 (ergocalciferol) is found in some plants and mushrooms. Many indigenous Arctic peoples traditionally ate marine animal livers and lichen — a symbiotic algae-bacteria organism — for their vitamin D. And of course, we get vitamin D (also in the form of cholecalciferol) from sunshine.
Regardless of the source, our bodies need to convert vitamin D into a bioactive form — a form that they can use.
This takes three steps.
- First, in the GI tract, cholesterol is converted to pro-vitamin D3 (7-dehydrocholesterol), which is then transported to the skin, where UVB radiation converts it to vitamin D3 (cholecalciferol).
- Our liver hydroxylates (adds an oxygen-hydrogen pair to) D2 or D3, so that they become 25(OH)D (calcidiol or calcifediol). This is the form of vitamin D that we can store in our tissues such as muscle or body fat (adipose tissue).
- Then, our kidneys convert 25(OH)D to its active form: 1,25(OH)2D (calcitriol or calciferol). (Other cells can do this as well, such as cells of our immune system.)
To do steps 2 and 3, our liver and kidneys use the enzymes 25-hydroxylase and 1-α-hydroxylase, respectively.
Once vitamin D is made into 1,25(OH)2D, it can affect the activity of over 900 genes. 1,25(OH)2D that is circulating in our bloodstream can pass through the plasma membrane of cells, and bind to the vitamin D receptor (VDR) in the cytoplasm. The VDR is a nuclear receptor, which means it’s then transported into the nucleus, where it binds to DNA to affect the expression of genes.
What vitamin D does
1,25(OH)2D has lots of jobs. For instance:
- It can affect the metabolism of skeletal muscle and protein synthesis, influencing how well we build muscle and recover from our workouts.
- It affects how calcium and phosphate move across the sarcolemma, the sheath of our muscle fibers, influencing muscular contraction and fueling.
- It upregulates insulin-like growth factor (IGF-1), which plays a role in anabolism and recovery. (We’ll look more at IGF-1 in Chapter 11, when we look at exercise and muscle performance.)
- It can regulate our immune system, helping us fight off pathogens such as viruses or bacteria.
- It can affect programmed cell death (apoptosis). This is a normal part of a cell’s life cycle that kills dysfunctional cells.
- It’s involved in the transport and absorption of minerals like calcium, magnesium, iron, phosphate and zinc, all of which can affect cell signaling, tissue repair, and bone health.
- It can strengthen tight junctions, the seals between cells such as our skin cells or intestinal lining cells (much like mortar between bricks), which also prevent pathogens from getting in.
- It helps to regulate genes involved in glucose and lipid (fat) metabolism. Low vitamin D is correlated with metabolic problems such as insulin resistance or hypertension.
- It’s involved in the health of our reproductive system. Low vitamin D is linked to diseases such as polycystic ovarian syndrome (PCOS), ovarian and breast cancer, and endometriosis. In men, vitamin D levels are correlated with testosterone levels.
Thus, how our bodies process and use vitamin D significantly affects our health and fitness.
People whose vitamin D is low might not recover, build muscle, or fight off infections as well as those with more vitamin D. People with low vitamin D may also feel more pain and have more inflammation. (By the way, this doesn’t necessarily mean that low vitamin D is the cause of all of these things. It may be a correlation or a secondary effect.)
Genetic variations in using vitamin D
If you understand the basics of how vitamin D works, you can probably imagine that genetic variation could affect many components of vitamin D metabolism, as well as vitamin D’s ability to influence genetic expression.
Here are some examples of how this might work.
Cholesterol synthesis polymorphisms
We’ll learn more about cholesterol below, but since vitamin D is part of the family of “ster” molecules that are related to cholesterol, variations in genes involved in cholesterol metabolism can also affect vitamin D metabolism.
The gene DHCR7 codes for the 7-dehydrocholesterol reductase enzyme, which converts 7-dehydrocholesterol (7-DHC) to cholesterol. Since both cholesterol and vitamin D can be synthesized from 7-dehydrocholesterol, a loss of function mutation in DHCR7 can interrupt vitamin D synthesis.
Hydroxylase polymorphisms
We might differ genetically in how well our bodies are able to make hydroxlases — the family of enzymes that (among other jobs) convert vitamin D2 and D3 to the form of vitamin D that we can store and use.
Variations in genes coding for cytochrome p450 (CYP) enzymes (such as CYP3A4, CYP2R1, CYP24A1, or CYP27) can affect how much vitamin D we naturally have. People with mutations in these genes may not make enough vitamin D even if they eat well and get enough sunshine.
Vitamin D receptor (VDR) polymorphisms
Once bioavailable vitamin D enters the cell, it binds to the VDR. There are several VDR polymorphisms that affect gene expression, and are linked to many metabolic diseases (such as cancer, diabetes or heart disease), infectious diseases (such as tuberculosis), autoimmune diseases (such as multiple sclerosis or asthma), and chronic inflammation.
For instance, the FokI variation (rs10735810) can change the length of the VDR protein, which can then change how it works. Other VDR polymorphisms such as the ApaI variation (rs7975232), the TaqI variation (rs731236) and the BsmI variation (rs1544410) are linked to a higher risk of bone and connective tissue disorders (such as spinal disc degeneration, low bone density, and fractures) as well as other chronic diseases.
One handy thing about many of these VDR variations: Unlike many genetic variations, they’ve been studied in non-European populations (such as people of Iranian, Chinese Kazakh, Gujarati, Indonesian, and Kenyan descent). This gives us some useful data about what these variants’ effects might be in different groups of people.
Vitamin D binding protein polymorphisms
To have effects on tissues throughout the body, vitamin D has to get to them.
The GC gene codes for the vitamin D binding protein (DBP), otherwise known as Gc-globulin. This protein binds to and transports vitamin D.
Gc-globulin is part of our body’s response to injury. It can activate macrophages (a type of immune system cell that’s part of our “cleanup crew”) and bind to actin released by dying cells, preventing it from coagulating and gumming up the works. We’ll learn more about actin in Chapter 11 on exercise and muscle performance.
There are 3 common variants of GC (Gc1F, Gc1S and Gc2), along with over 120 rarer variants. (Because these variants are relatively infrequent, they can help us explore heredity and links between global populations.) People of European descent tend to have Gc2, which is rare in people of African descent, who typically have Gc1f instead.
Variations in GC (and hence DBP / Gc-globulin) have been linked to risk of (or resistance to) many types of diseases, such as osteoporosis, thyroid disease, diabetes, cardiovascular disease, multiple sclerosis, and more.
What this means for you
- Blood levels of vitamin D are strongly linked to health and disease, as well as athletic performance and fitness. Typically, vitamin D deficiency is correlated with poorer health and 23andMe recovery.
- Given the complexity of vitamin D metabolism, it’s entirely likely that you might have a genetic polymorphism that affects your vitamin D levels. The effect of having this genetic variant may range from significant to minor.
- Genetic testing may give you useful information about your potential vitamin D levels and/or risk of deficiency.
- 23andMe tests for several dozen VDR SNPs, though it doesn’t include any predictions about vitamin D levels in its trait or health condition lists.
- The Nutrigenomix test kit analyzes CYP2R1 (rs10741657) and GC (rs2282679) variations to assess risk for vitamin D insufficiency.
- It may be simpler and more straightforward to simply do a vitamin D lab test to assess your current blood levels.
- Based on your lab test, you can then work with a nutrition professional to decide whether vitamin D supplementation (or getting out in the sunshine) is appropriate.
Thyroid function
Our thyroid, which hugs our windpipe, is an endocrine gland that helps regulate many important physiological processes.
Our hypothalamus secretes thyrotropin-releasing hormone (TRH), which then stimulates the pituitary to release thyrotropin (aka thyroid-stimulating hormone, or TSH).
In turn, this stimulates the production and synthesis of hormones like calcitonin, triiodothyronine (T3) and thyroxine (T4), which act elsewhere in the body to help regulate:
- basal metabolic rate (BMR);
- gut motility;
- digestion, absorption, and use of nutrients;
- cardiovascular and respiratory function;
- energy use and storage;
- development;
- sexual and reproductive functions;
- sleep;
- moods; and
- calcium metabolism (which includes bone health and density).
Importantly, T3 also acts on mitochondrial and nuclear DNA to regulate gene expression through binding to THR and activating transcription.
Hypothyroidism and autoimmunity
Many of our Precision Nutrition clients, particularly women, come to us frustrated because they can’t seem to lose fat. Or their digestion is sluggish. Or they don’t seem to have the energy they used to have.
In many cases, part of the problem is hypothyroidism — their thyroid has “slowed down”, and thus their metabolism has too. (The opposite is hyperthyroidism, an over-active thyroid that “speeds things up”.)
Environmental factors (such as chronic dieting) can play a role in thyroid problems, but so can genetic factors, for example, genetic variants that increase a person’s risk of autoimmune thyroid diseases (AITD) such as Graves’ disease or Hashimoto’s thyroiditis.
In AITD, as with other autoimmune diseases, the body’s immune system attacks and slowly destroys healthy tissue — in this case, the thyroid gland. Over time, the thyroid is unable to produce the hormones it needs to support a healthy metabolism.
Some estimates suggest that AITD affects about 5% of the American population, and perhaps as many as 10-20% of all women in their lifetime.
While AITD can be inherited, studies using twins and family members show that environmental factors and stressors also play a role.
Genes related to AITD risk fall into three general groups:
- The human leukocyte antigen (HLA) gene complex that codes for major histocompatibility complex (MHC) proteins in humans. These cell surface proteins regulate our immune system by helping our cells recognize foreign molecules. (We’ll look at HLA genes more when we look at food sensitivities.)
- Non-HLA immune-related genes such as CD40, CTLA-4, PTPN-22, FOXP3, and CD25, which code for other proteins of our immune system.
- Thyroid-specific genes, such as:
- TG, which codes for thyroglobulin proteins.
- TSHR, which codes for a receptor that binds to thyroid stimulating hormone (TSH). Second to HLA-related genes, TSHR is the gene most closely linked to the risk of Graves’ disease.
- TPO, which codes for thyroid peroxidase, an enzyme that helps produce the thyroid hormones T3 and T4.
Some evidence also suggests that other factors, such as variants in genes coding for our vitamin D receptors (there they are again!), may play a role.
Let’s look at a few SNPs that 23andMe tests for.
PTPN22
The PTPN22 gene codes for the protein tyrosine phosphatase, non-receptor type 22, a protein expressed mostly in lymphoid, or immune system, tissues. Variations in this gene are associated with autoimmune disorders such as rheumatoid arthritis, multiple sclerosis, Type 1 diabetes, and/or Crohn’s disease.
23andMe tests for a SNP on this gene known as rs2476601.
Research on European subjects found that each copy of an adenine (A) at this SNP increases a person’s odds of hypothyroidism by 1.36 times, and increases their odds of developing Hashimoto’s thyroiditis by 1.7 times.
Other research testing PTPN22 variations in a Korean population didn’t find the same results, but another SNP (rs12730735) and a particular haplotype were both associated with the risk of autoimmune thyroiditis. Similar results were found in a Chinese population. In a Japanese population, PTPN22 doesn’t seem to have any connection at all to AITD, though having a particular haplotype seems to offer some protection against the disease.
In a Jordanian population, researchers did not find that rs2476601 played a role, perhaps because the SNP doesn’t often appear in this group. However, it was found in an Egyptian population, and did increase the risk of AITD.
Thus, the contribution of rs2476601 may vary by ethnicity.
FOXE1
Forkhead box (FOX) proteins are typically involved in regulating cell growth, development, differentiation, and survival. FOXE1 codes for forkhead box protein E1, a protein that helps develop the physical structure of the thyroid.
Mutations in this gene cause Bamforth–Lazarus syndrome (a rare disease in which thyroid tissue is underdeveloped or missing entirely) and congenital hypothyroidism. FOXE1 has been associated with TSH levels, hypothyroidism, and thyroid cancer.
Research in a European population found that people with the guanine-guanine (GG) genotype at rs7850258 in the FOXE1 gene were 1.35 times more likely to have primary hypothyroidism compared to those with the adenine-guanine (AG) genotype, whereas people with the adenine-adenine (AA) genotype have a slightly lower risk.
Another SNP near the FOXE1 gene (rs755109) was found to be associated with TSH levels in an isolated Pacific Island population.
SH2B3
The SH2B3 gene codes for the Src homology 2-B 3 (SH2B3) protein, which is part of the SH2B family of proteins. These proteins are found in many types of tissues and cells.
In particular, SH2B3 regulates signaling related to hematopoiesis (the formation of blood cells), inflammation, and cell migration (the programmed movement of cells to specific locations).
As with PTPN22, mutations of SH2B3 are related to autoimmune diseases such as celiac disease and Type 1 diabetes (T1D). Thyroid disease may involve autoantibodies against thyroid peroxidase (TPO). In both PTPN22 and SH2B3, variants associated with an increased risk of Type 1 diabetes were also linked to having TPO antibodies.
23andMe tests for a SNP called rs3184504. In people of European descent, having the thymine-thymine (TT) genotype slightly increased the risk of having hypothyroidism, while having the cytosine-cytosine (CC) genotype lowered the risk slightly.
Another study, also in Europeans, showed a link between the rs653178 SNP and Graves’ disease.
VAV3
VAV3 codes for a protein (guanine nucleotide exchange factor VAV3) that regulates the Rho family of proteins, which regulate a wide range of cellular activities, such as signal transduction. You can think of Rho proteins as cogs in a communication system that allows your cells to respond properly to external and internal changes.
VAV3, like other genes related to AITD, is involved in immune function.
According to 23andMe studies, in populations of European descent:
- Each copy of a C at rs4915077 in the VAV3 gene was associated with 1.3 times the odds of hypothyroidism.
- People with the GG genotype at rs2517532 had 1.16 times higher odds of hypothyroidism compared to those with the AG genotype. Those with the AA genotype had 1.16 times lower odds of hypothyroidism.
What we found in our sample
We asked people whether they’d been diagnosed as hypothyroid.
Four people had. So we looked at their genetic data to see how they stacked up. We also looked at the people who should (by the genetic prediction) have been at a higher risk.
Our sample is small, so it’s hard to draw conclusions. But we did find that these particular SNPs didn’t generally have strong predictive value.
Many people who “should” have hypothyroid symptoms didn’t. Conversely, people who had diagnosed hypothyroid disease didn’t have any known genetic variants that might have increased their risk.
PTPN22 rs2476601
Each copy of an adenine (A) in a European population should theoretically increase a person’s risk of hypothyroidism 1.36 times, and their odds of developing Hashimoto’s thyroiditis by 1.7 times.
Yet we had only 1 adenine-guanine (AG) among our diagnosed hypothyroid group; the rest were guanine-guanine (GGs) — in other words, low-risk.
In this sample, we had no highest-risk AAs, but we did have 5 other AGs who seemed perfectly healthy.
FOXE1 rs7850258
People with the GG (guanine-guanine) genotype at this SNP in the FOXE1 gene were 1.35 times more likely to have primary hypothyroidism compared to those with the AG (adenine-guanine) genotype.
In our sample, 2 people with GG had hypothyroidism, while 2 had the AG.
But again, 12 other people had GG without hypothyroid symptoms.
SH2B3 rs3184504
Having the TT (thymine-thymine) variant of this SNP slightly increased the risk of having hypothyroidism, while having the CC (cytosine-cytosine) genotype lowered the risk slightly.
In our hypothyroid sample, we had 2 CCs and 2 CTs, plus two other healthy TTs.
VAV3 rs2517532 and rs4915077
Each copy of a C at rs4915077 in the VAV3 gene was associated with 1.3 times the odds of hypothyroidism.
In our sample, no person with hypothyroidism had a C. All were TT.
People with the GG genotype at rs2517532 had 1.16 times higher odds of hypothyroidism compared to those with the AG genotype, and those with the AA genotype had 1.16 times lower odds of hypothyroidism.
In our sample, 2 had AG (moderate risk) and two had GG (highest risk). 9 other healthy people also had the higher-risk GG.
What this means for you
- Thyroid disease doesn’t just affect our thyroid. It can also affect what is “downstream”, such as other metabolic processes.
- There is a strong genetic risk component to thyroid disease. Further, genetic risk factors for thyroid disease are genetic risk factors for other (autoimmune) conditions. If thyroid disease or other autoimmune conditions run in your family, consider genetic testing to alert you to any potential risk.
- Your specific genetic risk may vary by ethnicity. This might mean a different gene variant is more significant than another, or that thyroid disease may emerge from related but distinct pathways.
- Even if you have genetic risk factors, it doesn’t necessarily mean you will get a given disease.
- Consider the big picture of other contributing factors. For instance, the prevalence of AITD varies significantly by geography, from 448 cases per 100,000 in Scotland to 273/100,000 in Western Australia, to 27/100,000 in Spain. Since these countries all have a large population of white European descent, there are clearly other environmental components at work.
Glucose, insulin and metabolic syndrome
Glucose, a simple sugar or monosaccharide, is the raw material for building ATP, our cells’ energy.
Normally, our bodies maintain glucose homeostasis. In other words, we balance our blood sugar within a relatively narrow range.
If we eat carbohydrates (say, bread or rice), they break down into glucose when we digest them. Our pancreas then releases insulin to transport the glucose into our cells, to be eventually made into ATP or stored as glycogen or fat for later use.
This process is tightly regulated. Our bodies sense the presence of glucose; insulin is released as needed; and glucose is stored in cells. When glucose drops too low, we release stored fuel and our brain sends us the signal to eat.
Both insulin production (i.e., how well we can make insulin) and insulin resistance (i.e., whether our cells respond appropriately to insulin) are heritable.
Insulin helps signal glucose transporters (GLUTs), particularly GLUT4, to move glucose into cells. When insulin doesn’t work properly, we may have both higher circulating levels of blood sugar / glucose and insulin. This can eventually damage tissues.
So we want insulin sensitivity to be high — in other words, we want our cells to be able to “hear” insulin’s signals, and respond.
Metabolic syndrome
If our blood sugar is consistently higher than normal, this signals a metabolic problem. We may be in the early stages of prediabetes, or even full-blown Type 2 diabetes (T2D).
This situation, and other phenomena that go with it (such as high blood pressure, altered blood lipids, fatty deposits in and around internal organs such as liver or kidneys) are collectively known as metabolic syndrome. (We’ll look at cholesterol and triglycerides more below.)
Metabolic syndrome is a “disease of affluence”, and currently one of the biggest health problems worldwide. Populations that may have avoided it a few decades ago (such as in mainland China or many parts of sub-Saharan Africa) are now confronting its health effects.
Metabolic syndrome is a complex health problem that involves many processes and tissues. Over time, almost the entire body can be affected in some way.
For example:
- Nerves may be damaged (aka neuropathy) along with blood vessels.
- Fat may infiltrate our liver, kidney, muscles, heart, and other organs.
- Our immune system may become over-active, and we may have chronic inflammation.
- Our gut motility and microbiota may change.
- Our eyes may deteriorate.
- Our skin and connective tissues may break down.
Like other complex health conditions, there is no single “metabolic syndrome gene”.
Obesity susceptibility genes and dietary carbohydrates
Typically, metabolic syndrome goes along with higher levels of body fat, especially around the midsection or in the abdominal cavity (aka visceral fat).
Along with being a storage depot for excess energy, adipose (fat) tissue is part of our endocrine system: It secretes hormones (such as estrogen or leptin) along with other cell signals and messengers.
Adipose tissue is closely linked to insulin sensitivity / resistance, and hence, our risk of T2D. Yet researchers have wondered:
How are some people metabolically healthier than others, even with the same amount of body fat?
Our genetic makeup is one factor.
For instance, through its effects on the AMPK signaling pathway, the fat-secreted hormone adiponectin plays an important role in insulin sensitivity by helping our muscles and liver oxidize fatty acids and take up glucose. (This is good.) When we have a lot of adipose tissue, adiponectin tends to go down. (This is not so good.)
Yet people may have genetic variants in ADIPOQ, the adiponectin gene, that change their risk of metabolic disease. For instance, in some populations (such as East Asians), having the GT/TT version of the rs1501299 SNP (rather than GG) is associated with a higher rate of metabolic disorders such as fatty liver or insulin resistance.
So, our risk for metabolic disease may depend not just on the absolute amount of adipose tissue we have (though past a certain point, even the luckiest combination of genes can’t protect us from the health effects of extremely low or high amounts of body fat) but on our genetic makeup as well.
We’ll look more at body fat in the next chapter.
For now, here are the basic concepts:
- Many genes linked to body weight and fatness are also involved in glucose metabolism.
- In general, gene variants associated with higher body weight or more body fat are also linked to weight gain when people eat a high-carbohydrate diet.
Importantly, this doesn’t mean that carbohydrates are “bad”.
Or that glucose makes us obese.
Or that insulin is a silent but deadly killer (as is often claimed by promoters of low-carb fad diets).
Let’s take another perspective:
Our genetic makeup may make us more likely to respond in certain ways to certain nutrients.
This is especially true with processed carbohydrates, since many processed foods make a lot of sugar available in a form that is digested very quickly (which means that a lot of sugar hits our system all at once).
Some research suggests that genes that may make us more susceptible to obesity also affect how our bodies respond to dietary carbohydrates. Importantly, this response often isn’t just about carbohydrates, but also about how our bodies manage and mobilize fats. (More on this below.)
Also bear in mind: Research here is often somewhat contradictory. It’s not as simple as saying “More sugar is bad.”
PLIN and ADRB2 genes
For instance, there is a complex relationship between carbohydrate intake, body fatness, and the family of PLIN genes, which code for perilipin (PLIN).
In our fat cells (adipocytes), we have a lipid droplet — essentially an oily bubble of stored fat.
Perilipin surrounds these lipid droplets, and helps control the metabolism of adipocytes by regulating how fat-mobilizing enzymes (lipases) interact with the stored fat.
Since the job of lipases is to break down fats so they can be used for energy, perilipin surrounds the lipid droplet like a protective wall, and ensures that it doesn’t get randomly digested.
Our β-adrenergic receptors respond to catecholamine hormones like epinephrine (adrenaline), and activate processes to help us free up stored energy, get our muscles moving, and do other autonomic nervous system jobs.
Three genes code for β-adrenoreceptors: ADRB1, ADRB2 and ADRB3.
These genes and their variants vary by ethnicity, which can (along with many other genetic factors, of course) affect how lean or fat you may “naturally” be.
When certain β-adrenergic receptors are activated (for example, during exercise), they also tell our bodies to start making stored fat available so that we can use it. Once β-adrenergic receptors get the word that it’s go time, they tell perilipin to change shape — to roll back the gate protecting the lipid droplet, and expose the stored fat to lipases that can start breaking it down for fuel.
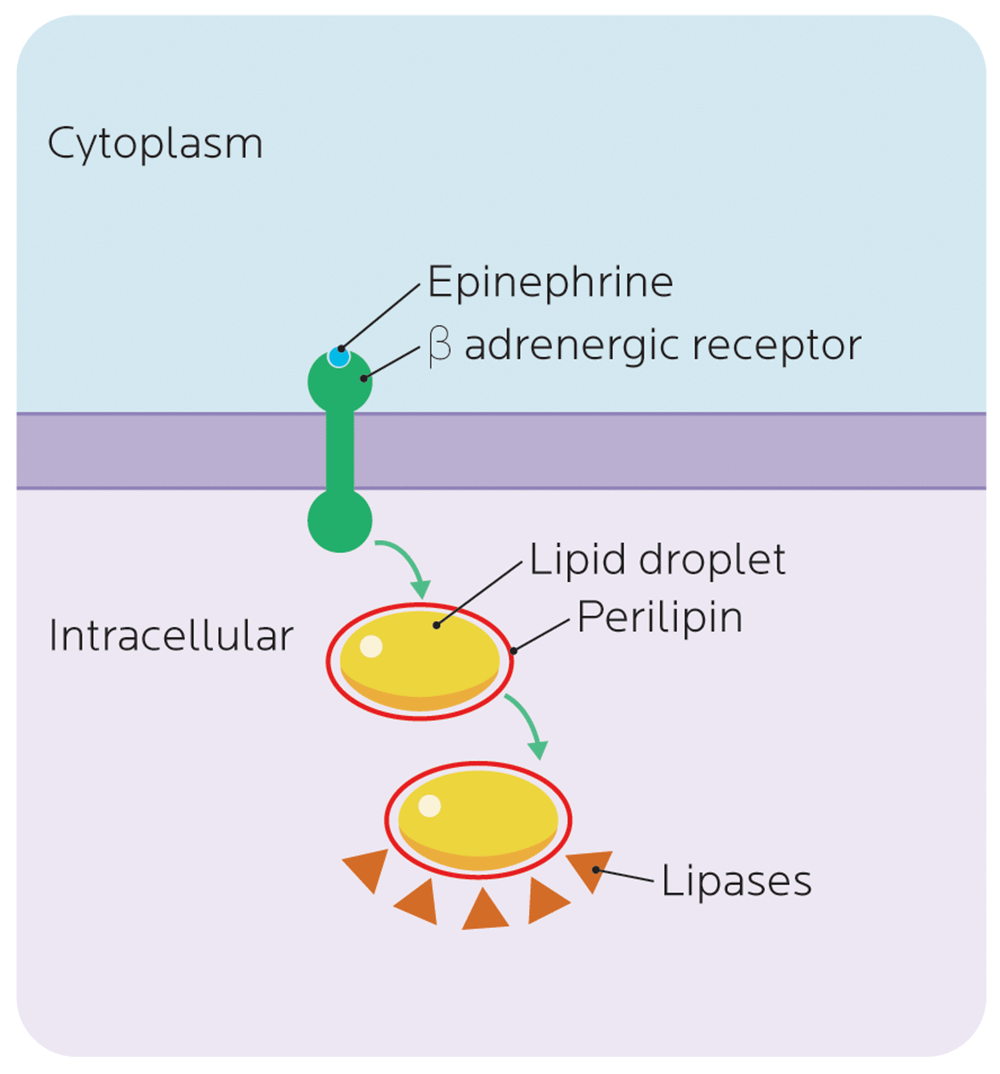
In general, we usually want our stored fat to be easily available. However, with some variations of the PLIN genes, which code for perilipin, the perilipin may be less responsive to the signal to open the gate. Fat stays locked behind the protective wall.
Conversely, rare genetic mutations of PLIN genes may result in lipodystrophy, disorders of fat metabolism that result in wasting of fat stores, or fat deposits in unusual places (such as on the hands).
In a study of Hispanic people of Caribbean origin, researchers found that when participants with a particular variant of the PLIN gene (PLIN 11482 G > A) ate more complex, higher-fiber carbs, they were leaner; when they ate fewer complex carbs, they were more likely to be obese. Interestingly, at least in this study, simple sugars or total carbohydrates didn’t seem to have any relationship to body fatness, nor did other PLIN variants.
Other studies have confirmed that PLIN variants predict both metabolic syndrome and weight loss.
Similar results with some PLIN variants and insulin resistance were found in a Singaporean population made up of Chinese, Malay, and South Asians. These variants of the PLIN genes may be old ones, present before human populations diverged.
The association between specific PLIN gene variants and fatness also seemed related to fat intake, particularly saturated fats — when people with two copies of a PLIN variant consumed carbohydrates and saturated fats, they were significantly more likely to have insulin resistance, a precursor to Type 2 diabetes.
Other genes related to obesity susceptibility, such as FAIM2, FLJ35779, FTO, LRRN6C, RBJ, and SEC16B also seem to interact with dietary carbohydrates to increase BMI.
What this means for you
Studies on human diets are generally self-reported (rather than carefully controlled, like in lab animals). Ways of measuring dietary responses also vary.
So we have to be careful about how we interpret results from any single study.
Nevertheless, the findings suggest:
- Not all bodies respond the same way to the same diet. Your friend may thrive on a low-carb, high-fat diet (or a high-carb, low-fat diet), whereas you may find yourself feeling and performing worse when you try the same thing.
- There’s no one-size-fits-all diet plan that will work for everyone, all the time. It’s important to test, observe, and assess (based on evidence) what nutrition protocols work best for your unique body.
- Genetic testing can give us some clues, but not a detailed prescription, for your “best diet”. We still don’t completely understand the complex relationship between genetic variants and metabolic health. Genetic testing may suggest particular dietary strategies that might help you make wise dietary choices for your physiological needs.
- Understanding the impact of genetics on metabolism helps you make informed decisions about your diet. At the very least, genetic testing may help explain why a particular diet may affect your health or physical performance. At best, you may find a diet that matches your “metabolic strengths” (i.e., what your body prefers).
- Processed sugars seem to cause the most metabolic problems, regardless of specific genetic variations.
Fasting glucose predictions
What predicts our risk of Type 2 diabetes (T2D)?
We know that T2D is correlated with many physiological and lifestyle factors, such as:
- having more body fat (and less lean mass);
- being sedentary; and
- eating a diet with lots of processed foods.
We also know that adipose (fat) tissue is a hormonally active tissue.
Early studies on Type 2 diabetes (T2D) often used people with more body fat, who were more likely to develop T2D. It wasn’t always clear whether the progression of T2D was due to environmental effects (for instance, a high-sugar diet), having a certain amount of body fat, or an underlying genetic predisposition (in other words, what someone was born with).
Later studies looked at relatively leaner people to account for the environmental component of T2D, or for the role that adipose tissue gained in later life might play.
One way to test for insulin resistance and prediabetes is with fasting glucose: measuring blood sugar levels after a person hasn’t eaten for several hours.
Alhough research suggests that genetic variants linked to higher fasting glucose don’t always correlate neatly with T2B risk, there’s a lot of overlap and fasting glucose is still a pretty good predictor.
G6PC2
23andMe looks at a SNP known as rs560887, which is associated with fasting blood glucose levels in European populations.
This SNP appears in the G6PC2 gene, which codes for glucose-6-phosphatase catalytic subunit-related protein (also known as IGRP), a protein that is expressed in islet cells of the pancreas.
This SNP is associated with the function of pancreatic beta cells, which store and release insulin, as well as C-peptide and amylin (which helps control how quickly glucose is released into the bloodstream).
In the study used by 23andMe, each T at rs560887 lowered subjects’ fasting plasma glucose level by 0.06 mmol/L (1 mg/dl).
Allele | Average fasting glucose levels |
CC | 5.18 mmol/L (about 93 mg/dl) |
CT | 5.12 mmol/L (92 mg/dl) |
TT | 5.06 mmol/L (91 mg/dl) |
For reference, a fasting blood glucose level lower than 5.6 mmol/L is considered normal, while anything over that is considered prediabetic.
What we found in our sample
We had some blood tests lying around, so we looked for correlations.
One person from of our PN sample had just been diagnosed with prediabetes. Yet according to the G6PC2 SNP, with a CC profile, they should have had lower than average blood sugar.
We also had 2 TTs, who were predicted to have higher than average blood sugar but whom, to date, seem healthy.
Not surprisingly in our health-and-fitness-oriented population, many CT people had normal or lower than average fasting blood glucose. This suggests that lifestyle choices play a bigger part than a single SNP.
What this means for you
- Blood glucose is an important health indicator. Don’t rely only on a genetic test. At best, a genetic test can only predict possible risk. It can’t tell you what your blood glucose actually looks like. While blood glucose normally rises and falls as we eat and fast between meals, chronically elevated blood sugar can tell you that there is an underlying health problem.
- Get regular bloodwork done. Generally, by the time fasting glucose is affected, people may be well on their way to metabolic syndrome. So having your fasting glucose tested, along with your blood lipids (see below) as part of your regular medical checkup (for instance, annually) is a good idea, especially as you age.
- If you are a do-it-yourself kind of person, you can buy a home glucose monitor and test your glucose throughout the day, including after meals. Postprandial — “after meals” — blood sugar levels will give you a better idea, sooner, of what your blood glucose is up to, compared to fasting glucose.
- If you’d like to improve your overall wellness, see our strategies for healthy metabolism in Chapter 12.
Blood lipids and lipoproteins
Once we eat and digest foods containing dietary fats, these fats (aka lipids) are packaged into various formats (such as triglycerides) to be transported around the body and used.
Much like blood glucose, levels of these blood triglycerides and lipoproteins (proteins that transport lipids) are important indicators of metabolic health. (We’ll look more closely at lipids, triglycerides, and lipoproteins below.)
As with glucose, it’s normal to have some circulating lipids and lipoproteins, but we don’t want too many, too often.
Lipid and lipoprotein levels are strongly influenced by how much body fat we have along with our environment and our choices, such as our nutrition, our activity, smoking or drinking, or other medications. They can also be influenced by genetic factors.
Everyone has that proverbial great-aunt who lived to be 103 and swore by her daily whiskey and buttered bacon sandwich. And as we witness this fiercely unrepentant and apparently immortal boozing, buttering, and bacon-ing, we ask ourselves, Why am I eating all this damn healthy food anyway?
On one level, that’s a good question.
Some people seem able to consume “unhealthy” food, drink like a fish, smoke a pack of cigarettes a day, and make it to triple digits completely unfazed.
Other people seem to do everything “right” and get taken out by a heart attack or some other metabolic disease far too early.
Frankly, it seems deeply unfair sometimes.
And, indeed, it may be unfair, in the sense that some people may be naturally better at managing their blood lipids than others. This relative advantage may, in part, be due to genetic factors.
The genetics of lipids
To date, we know of about 160 genetic variants associated with lipid levels.
Of course, not all are equally important or strongly linked. Some operate mainly in particular processes (such as regulating the expression of other genes) or particular tissues (such as the liver).
We still don’t fully know how all genetic variants interact with blood lipids and other relevant biomarkers.
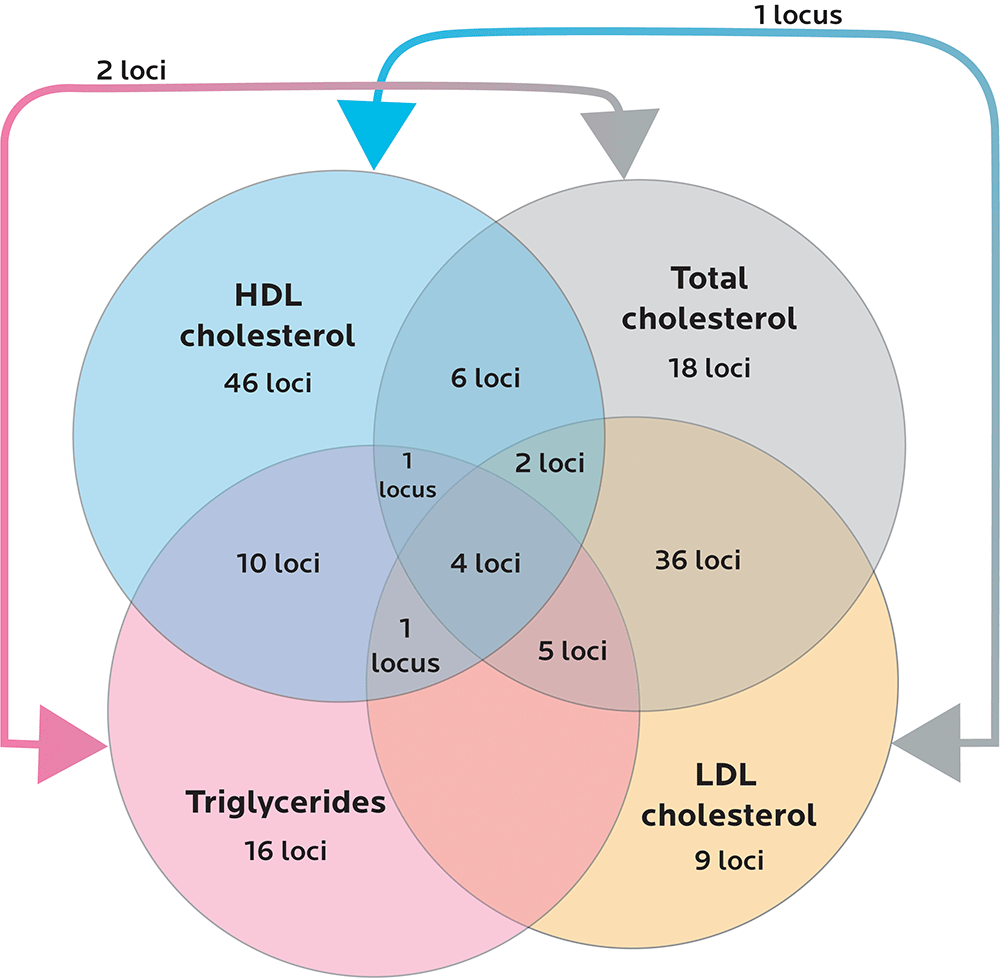
Diagram adapted from Willer CJ, Schmidt EM, Sengupta S, et al. Discovery and Refinement of Loci Associated with Lipid Levels. Nature Genetics. 2013;45(11):1274-1283. doi:10.1038/ng.2797.
Lipoproteins and the basic blood lipid panel
If you go to your doctor and get a “cholesterol test” done, it’s helpful to understand what this really means.
Cholesterol is a waxy lipid (imagine something like lard). We make cholesterol in our liver, but it’s also in our food. We use cholesterol to make many important molecules that our bodies use, including our sex hormones.
Triglycerides are molecules of fat made of three fatty acids hooked to a glycerol backbone. When we digest fat, our bodies package fatty acids into this format, and transport them through the bloodstream to be stored in adipose cells.
Lipoproteins are proteins that transport lipids. Oil and water don’t mix, and neither do lipids and blood. Fats have to hitch a ride on lipoproteins, like a bunch of passengers on a little inner tube floating down a river. Lipoproteins come in different sizes, amounts, and densities. Density refers to the fat-to-protein ratio: When there’s more fat and less protein, the particles are considered less dense, and they become more buoyant.
Apolipoproteins are protein-based components of lipoproteins. They’re encoded by genes that start with the letters APO (such as APOB or APOE). We’ll look at apolipoproteins a little later.
We can divide lipoproteins into a few general categories based on density:
- HDL-C is high-density lipoprotein, aka the “good cholesterol”. This brings cholesterol back to the liver for recycling.
- LDL-C is low-density lipoprotein, aka the “bad cholesterol”. This transports cholesterol away from the liver to elsewhere in the body, and can be part of the process of inflammation that underlies cardiovascular disease risk.
- IDLs, or intermediate-density lipoproteins, somewhere between LDL and VLDL particles.
- VLDL is very low-density lipoprotein, also a kind of “bad cholesterol”. We also want this to be lower.
- Chylomicrons, also known as ultra-low-density lipoproteins (ULDLs).
When we get a basic blood lipid panel done at our doctor’s appointment, it will most commonly measure:
- Blood triglycerides: how much total triglyceride is in all the lipoprotein particles combined.
- Total cholesterol: how much cholesterol is in all the lipoprotein particles combined.
- HDL-C: how much HDL-C we have.
- LDL-C: how much LDL-C we have.
Generally, we want most of these to be lower, except for HDL, which we want to be relatively higher.
Having too many triglycerides circulating in our blood is known as hypertriglyceridemia. Very high cholesterol levels are known as hypercholesterolemia. The combination of these, which creates a poor blood lipid profile, is known as dyslipidemia.
All of these are risk factors associated with metabolic syndrome and its associated diseases, although they don’t necessarily mean that someone will always develop health problems.
All of these risk factors can be affected by diet and exercise, along with other lifestyle choices and environmental factors… but can also be partially shaped by genetics.
For instance, research has found ethnic differences in the LPL and LIPC genes, which code for lipases (enzymes that help us break down fats) and are expressed in liver, heart, muscle and fat tissues.
Compared to people of European ancestry, people of African descent (including West African, Afro-Caribbean and African-American) were more likely to have particular lipase variants that protected them from coronary atherosclerosis (aka hardening of the arteries).
What this means for you
- Blood lipids are important health indicators. If you’d like to know what they are, measure them directly with a blood test.
- Don’t rely only on a genetic test. At best, a genetic test can only predict possible risk. It can’t tell you what your blood lipids actually look like.
- Get regular bloodwork done. Having your blood lipids tested along with your blood glucose (see above) as part of your regular medical checkup (perhaps annually) is a good idea.
- If you are diagnosed with dyslipidemia or other metabolic health concerns, discuss this with your health care professional. If you have heritable dyslipidemia, you should still be doing all the things that promote healthy metabolism (you know, the whole “Don’t smoke, keep a healthy body fat level, get some exercise” thing), but you may need additional medical support.
- Review our strategies for healthy metabolism in upcoming chapters.
Hypertriglyceridemia and zinc fingers
Normally, we want triglycerides to be stored safely in our fat cells, waiting to be used for energy. We don’t want too many of them roaming around our bloodstream for too long.
Some people are genetically more likely to have higher blood triglycerides, aka hypertriglyceridemia.
ZNF259 and rs964184
23andMe tests for a SNP known as rs964184 on the ZNF259 gene (which is also sometimes known as ZPR1).
The ZNF in this gene name stands for “zinc finger”.
While Zinc Finger would be a terrific band name (and, indeed, someone has already created a song that uses the primary structure of another ZNF protein), it actually refers to a zinc-based part of a protein that gives a protein a particular shape. (You’ll remember from Chapter 2 that the physical shape and structure of a protein affects how it works.)
Most proteins with zinc fingers are “interaction molecules” that are designed to bind to DNA, RNA, or other protein molecules. So their shape crucially affects how they are able to do this job.
The protein coded by ZNF259 binds proteins that are involved in insulin sensitivity, glucose and fat metabolism, and fat storage, such as peroxisome proliferator-activated receptor gamma (PPAR-γ) or hepatocyte nuclear factor 4α (HNF4A).
ZNF259 is also located close to another gene complex that is strongly linked to blood lipids: APOA5–A4-C3-A1. Variants in APOA5 affect metabolism of chylomicrons, VLDL-C, and HDL-C. (We’ll look at the APO gene family below.)
In one study of people of European descent who had hypertriglyceridemia, each copy of a G at the rs964184 SNP on ZNF259 increased people’s risk of hypertriglyceridemia by about 3.3 times.
Other research in Japanese and South Asian populations has found a similar relationship between ZNF259 and the overall risk of metabolic syndrome. In this case, having the G form of the rs964184 SNP was linked to having higher blood triglycerides along with higher fasting plasma glucose and lower HDL cholesterol.
This suggests, again, that many features of metabolic syndrome are related.
Hypercholesterolemia
Like hypertriglyceridemia, hypercholesterolemia (excessively high blood cholesterol) can be inherited, a condition known as familial hypercholesterolemia (FH). FH is a risk factor for metabolic syndrome and related health problems like cardiovascular disease.
FH happens when genetic variations affect our body’s ability to clear LDL effectively from the blood. Over time, LDL and its cholesterol passengers can build up in tissues such as our blood vessels or connective tissues.
The most common genetic cause of FH is variations in the LDLR gene. LDLR codes for the low-density lipoprotein receptor that determines whether LDL-C can bind to a cell, and then be transported into it for safe storage. About 80-90% of people with FH who are genetically tested show variations in this gene.
The second most common cause of FH (only about 5% of cases) is variations in the APOB gene. You’ll remember that APO genes code for apolipoproteins — in this case apolipoprotein B.
23andMe looks at two variations in the APOB gene that are linked to FH:
- R3500Q, found mainly in people with European ancestry.
- R3500W, found mainly in people of Asian ancestry.
What we found in our sample
Several PN team members sampled reported having had a total cholesterol test that was higher than average.
Yet none of these people had the genetic variants of APOB that suggested they should have this profile.
Interestingly, all of these people were fit, often leaner than average. This tells us that other factors are probably at work in these higher-than-average blood tests.
What this means for you
- Blood lipids are important health indicators. If you’d like to know what they are, have them measured directly with a blood test.
- Don’t rely only on a genetic test. At best, a genetic test can only predict possible risk. It can’t tell you what your blood lipids actually look like.
- Get regular bloodwork done. Having your blood lipids tested along with your blood glucose (see above) as part of your regular medical checkup (perhaps annually) is a good idea.
- If you discover hypertriglyceridemia, hypercholesterolemia, or other metabolic health concerns, discuss this with your health care professional. If you have a heritable condition, you should still be doing all the things that promote healthy metabolism (you know, the whole “Don’t smoke, keep a healthy body fat level, get some exercise” thing), but you may need additional medical support.
- Review our strategies for healthy metabolism in upcoming chapters.
Alzheimer’s disease
Alzheimer’s disease is a neurodegenerative disease in which brain cells and their connections slowly break down, while wastes, plaques, and other bits of crud (such as neurofibrillary tangles, which are sort of like the hairballs that clog up drains) build up.
(Here’s more reading on Alzheimer’s from our PN blog.)
Alzheimer’s is now sometimes called “diabetes of the brain”, since some researchers suggest that it may be related to the same types of dysregulated glucose and insulin mechanisms as we find in Type 2 diabetes.
Alzheimer’s is a frightening prospect, and this fear may affect our choice to have our DNA tested.
Many of us at PN researching genetic data asked our parents to take part. Some parents were game. Some parents were not.
The most common reason given?
“I don’t want to find out I’ll get Alzheimer’s or something.”
We fear neurodegeneration. Losing our mind and physical function in an irreversible, inexorable march is a scary prospect.
Our co-author Krista watched her once mentally-agile, fiercely self-reliant grandfather become confused, frail, and terrified as Alzheimer’s punched holes in his brain. Now she (and her mother) wonder: Who’s next?
Currently, more than 5 million people in the US alone are diagnosed with Alzheimer’s disease, and this number is expected to triple by 2050. 1 in 9 people over 65 has some form of neurodegeneration attributed to Alzheimer’s; over 1 in 3 people over 85 does. It’s one of the fastest-growing causes of death for older people.
Alzheimer’s does, indeed, run in families. But, much like Type 2 diabetes and other chronic diseases, while Alzheimer’s is a genetic risk, it is not a genetic destiny.
One of the known genetic risk factors in developing Alzheimer’s disease is our friend the apolipoprotein — in this case, a variant on the APOE gene, which codes for the protein apolipoprotein E, a cholesterol carrier found in the brain and elsewhere in the body.
The APOE protein comes in three forms: ε2, ε3, and ε4. (The “ε” is the Greek letter epsilon.)
The APO ε4 variant is a major risk factor for Alzheimer’s in many populations, and seems to span people of European, African, and East Asian descent. In fact, compared to the averages for both of those groups:
- Among people of African descent, one copy of the ε4 variant is associated with 1.5 times higher odds of developing Alzheimer’s; two copies is associated with about 4 times higher odds in people of African descent.
- Among people of East Asian descent, one copy of the ε4 variant is associated with 4 times higher odds; two copies is associated with 12 times higher odds.
While the APO ε4 variant is associated with a higher risk of developing Alzheimer’s, it doesn’t act alone. For instance, risk increases when — in addition to the APO ε4 variant — people also have:
- A particular variant of rs2373115, a SNP in the GAB2 gene, which codes for the growth factor receptor-bound protein 2 (GRB2). This protein is involved in signaling and communication within and between cells.
- A particular form of rs1799724, a SNP near the tumor necrosis factor (TNF) gene, associated with altered levels of beta-amyloid plaques (one of the particular plaque types linked to Alzheimer’s) in cerebrospinal fluid (CSF).
- Variants in genes that regulate polymerases, enzymes involved in DNA and RNA assembly. For instance, having an I-G-T haplotype of 3 SNPs in the POLD1 gene and the ApoE-ε4 variant almost doubles the risk of Alzheimer’s, compared to just having the ApoE-ε4 variant alone. The POLD gene, which codes for the protein polymerase delta 1, is also associated with colon cancer, deafness, and lipodystrophy.
What this means for you
- Maintain good overall health. Body health includes brain health.
- If you’re concerned about possible genetic risk, this is worth getting tested for. We still don’t have cures for Alzheimer’s and other neurodegenerative diseases yet, but it does help to act preventively and catch potential issues early.
- Understand your data clearly. Be sure to discuss all results with your doctor and/or genetic counsellor, to understand the findings and discover what you can do to lower your risk.
- Review our strategies for healthy metabolism in upcoming chapters.
What’s up next
Now that we have a basic understanding of how metabolism works, and a few genetic factors that may affect it, let’s look at how genetics can also influence our body size, shape, and fatness (or leanness).